The 12 principles of green chemistry were developed in the 1990s by chemists who wanted to address the impact of their activities in terms of safety and sustainability. These principles are still relevant today, with many laboratories and manufacturing facilities adopting them to improve safety and reduce the environmental impact of their chemical reactions. The best part is that green chemistry practices can be implemented anywhere, from small benchtop experiments to massive, industrial scales.
Going ‘Green’ in Chemistry
Chemistry has long been considered an inherently ‘dangerous’ science, with the public perception of a chemical laboratory being one filled with explosive chemicals and ionizing radiation. Indeed, history shows that chemicals have the potential to cause damage to life and property. From individuals like Marie Curie sacrificing health for research to industrial-scale environmental disasters like the Bhopal gas tragedy, the word ‘chemical’ undeniably carries with it some bad reputation.
In recent decades, we have a better understanding of chemicals, to the point where we can even predict reaction conditions and by-products. We understand that our actions—no matter how small—have both personal and global consequences. Furthermore, the economic benefits of going ‘green’ in chemistry cannot be understated. Apart from improving safety standards and removing the need for protective controls, green practices save costs by improving the efficiency of reactions while reducing the treatment and disposal of toxic by-products.
To provide an example, in the pharmaceutical industry, the overall cost of drug design and development averages $2.5 billion USD per successful drug in 2021. This translates to higher drug prices for consumers, hence every opportunity to reduce costs, even down to the chemical level, is a welcome endeavor. So without further ado, below are the 12 principles of green chemistry.
12 Principles of Green Chemistry
One: Waste Prevention
The first principle is to do with waste prevention; it’s better to prevent waste than have to treat and/or dispose of it after the reaction. Just as in the hierarchy of hazard controls, we should identify and remove inefficient reactions that generate an excessive amount of waste. The Environmental Impact Factor (E-Factor) is used to quantify the amount of waste generated per kilogram of product; a reaction that produces 2 kg of waste alongside 1 kg of product would have an E-Factor of 2. More waste means less product, which also needs to be treated and disposed of.
Apart from chemical waste prevention, time and effort can also be reduced by assessing the processes in place. Performing 5S exercises in the laboratory can make for a more efficient workplace, achieving more with less waste.
In a laboratory, an inefficient reaction process might mean an extra carboy of waste or an extra hour of heating. However, in an industrial setting, this can mean tankers of excess waste and high power usage. Chemical waste treatment and disposal is an expensive process, further adding to costs. Therefore waste prevention is an important consideration for every reaction.
Two: Atom Economy
Atom economy (AE) is another useful tool that allows us to calculate the efficiency of a reaction. It is taken to be the total atomic weight of the product against the total atomic weight of the substrates. An atom-efficient reaction is, therefore, one that has a relatively high AE value. A reaction with a low AE will consume a lot more resources as starting materials to yield less product, likely generating more waste.
The Wittig reaction, for example, is a Nobel prize-winning reaction. It is a reliable way to create alkenes and covers a wide scope of substrates, but thanks to the triphenylphosphine groups it isn’t atom efficient at all! In fact, it generates more than 20 times more waste than product by mass. On the other hand, we have reactions such as the extremely versatile Diels-Alder [4+2] cycloaddition. The direct addition of diene and dienophile means that its atom economy is 1:1.

Three: Less Hazardous Chemical Systems
Needless to say, reactions should be designed with safety in mind. After all, somebody (most likely yourself) will be carrying it out, therefore minimizing exposure to hazards—be it substrates or products—should be high up on that priority list! Tert-Butyllithium, for example, is an extremely versatile reagent for the deprotonation of activated carbons and amines. However, it also happens to be pyrophoric – it catches fire upon exposure to air. What this means is that every time a syringe is filled with it, it becomes a mini flamethrower, complete with orange flames shooting out the tip.
Wherever possible, pyrophoric bases are avoided, especially after numerous accidents causing serious injuries and deaths involving t-BuLi hs occurred. Furthermore, tBuLi use requires extra safety precautions (such as an air-free environment) and regulatory controls to be in place, making it a real pain to handle.

Four: Designing Safer Chemicals
Continuing on from the previous principle, chemical safety extends beyond the laboratory or factory. The final product should be designed to be as non-toxic as possible, even if it is not made for consumption. We must remember that a portion of these chemicals will eventually make their way into the environment, so minimizing their impact is beneficial to us. This is especially true of drug products that are designed to have an effect on the human body, even in minute quantities. Even metabolites of drugs after they have been excreted from the body can continue to affect the environment, therefore it is often a regulatory requirement for these to be mapped and studied.
Five: Safer Solvents and Auxiliaries
An area that often goes under the radar when designing reactions is the use of solvents and auxiliaries. To save on time and optimization, it can be tempting to simply use solvent systems that have been shown to work in literature. But many of these solvents tend to be organic compounds, which are usually a combination of toxic, flammable and corrosive. Minimizing or removing the use of organic solvents is a key area in green chemistry. Alternatives include ionic liquids, fluorous solvents, and even supercritical carbon dioxide.
Most of the time, solvent waste is just disposed of at the end of the reaction. But this doesn’t have to be the case! Where cost and/or safety is an issue, there are filters and membranes that are able to retrieve a portion of the solvent with a high enough purity, to be re-used.
Six: Design for Energy Efficiency
Often an afterthought in the chemistry laboratory, energy efficiency is important for sustainability. Energy-intensive reactions that require extremely high reactions are unfavorable from a green chemistry standpoint; there are often ways to reduce energy barriers. Furthermore, fossil fuel depletion and climate change are forcing us to invest in renewable energy technologies, and it is no different from chemical reactions. Biofuels, solar, wind, hydro and geothermal sources of energy and other sources of ‘clean’ energy currently account for roughly 20% of the world’s electricity generation2.

Seven: Use of Renewable Feedstocks
Apart from being used for electricity generation, fossil fuels are also used in many of the products being manufactured today, from plastics to pharmaceutical products. While the global shift toward renewable energy is gaining traction, equally important is the sustainable use of chemical feedstocks.
A shift to renewable feedstocks in chemical synthesis will be a necessity in the future as we face fossil fuel depletion, with biomass presenting a viable option. Many renewable materials can be obtained from living organisms, such as cellulose, starch, glycerol and vegetable oils.
Lignin is the major component of plant cell walls. In the pulp and paper industry, it is disposed of as waste in huge quantities, often burned off on-site to provide energy. New applications for lignin have been found, including as a feedstock for the production of vanillin, DMSO and humic acid, among other important industrial chemicals.
Another example is chitin, found in the shell walls of crustaceans. It is a byproduct of the seafood (nobody eats shells!) industry and is similarly burned off or buried in landfills. Green chemistry research being conducted on chitin derivatives shows that it can be used in a wide variety of applications, such as water purification3.
Eight: Reduce Derivatives
In organic synthesis, many blocking groups are added to molecules to protect functional groups. However, every extra derivatization step in a reaction leads to increased waste and decreased yields. It is important to note that processes in nature do not make use of protecting groups, preferring to alter reactivity through other mechanisms such as the use of elaborate catalysts4. While our understanding of synthesis is certainly lagging behind, we can attempt to reduce excessive derivatization or improve the efficiency of protection/deprotection.
To streamline otherwise inefficient reactions, a popular choice in green chemistry is to use catalysts. These can be transition metal-based, organic catalysts or even free radicals. Non-covalent derivatization is another method that can be used to improve the efficiency of such reactions. In such processes, intermolecular bonds are used to control reactivity instead of the classic covalently bonded protective groups. As a result, reactions can then take place under milder conditions.
Nine: Catalysis
Catalysts are substances that reduce the activation energy of a reaction, also being regenerated on the other side. This leads to improved yields and better efficiencies while reducing waste and saving time, leading to more streamlined processes. They can be considered to be a major cornerstone of green chemistry, as the employment of catalysts ticks almost all the boxes of safe and sustainable practices. The Haber-Bosch process—another Nobel Prize-winning reaction—is a famous example of catalysis, with an iron-based catalyst promoting the reaction of nitrogen and hydrogen to form ammonia, at a remarkable efficiency of 97%.
As mentioned in the previous principle, nature makes use of catalysts (also known as enzymes) to improve yields and remove the need for protecting groups, a major source of inefficiency. Many research groups are now attempting to follow in the footsteps of nature, borrowing the structure of some of these catalysts to enable biomimetic reactions.

Ten: Design for Degradation
With a large focus on green chemistry being the reduction of waste and waste generation, we can start at the beginning by designing biodegradable products. Much of our waste ends up in landfills or is dumped into the ocean, where they take hundreds or even thousands of years to break down. In the meantime, they interfere with ecosystems or even make it back into our water sources. Chlorofluorocarbons (CFCs) were once used as refrigeration agents due to their inertness and ease of production. However, CFCs were found to be extremely persistent, where they wreaked catalytic havoc on the ozone in our stratosphere.
While it isn’t simple to pinpoint the exact rate of biodegradation of chemicals (it took almost 40 years for the link between CFCs and ozone destruction to be made!), we can use past data to predict their persistence. Halogenated functional groups, quarternary carbons, tertiary amines and other extremely stable groups are avoided as final products as they persist in the atmosphere for decades. Rather, esters and amides that can undergo catalytic breakdown are now used as viable, shorter-lived replacements.
Eleven: Real-Time Analysis for Pollution Prevention
Analysis of data relating to the points outlined above is important for the development of ‘greener’ techniques. Real-time approaches are highly sought after in analytical chemistry research, where many processes rely on off-site pre-treatment of the sample before the actual analysis can be conducted. If data can instead be gathered at the source, we can monitor reactions and immediately react to prevent pollutants from escaping as soon as possible. This can help to avert accidents while saving energy and raw material, all of which are advantageous from a green chemistry perspective.
Twelve: Inherently Safer Chemistry for Accident Prevention
The 12th and final principle of green chemistry is perhaps the most important. While certain dangers come with working with chemicals, safety practices have improved considerably in the last few decades. With better hazard identification and detection technology, we have a better idea of both the short and long-term effects of exposure. That said, the best way to completely prevent accidents from occurring is by eliminating said hazards. In the future, our goal should be to remove or substitute the chemicals or processes that present serious hazards, so that laboratories and production sites are inherently safe places to work at.

Green Chemistry for a Greener World
Though these 12 principles provide a good reference when designing new chemicals, many newer reactions do not offer this sort of flexibility of optimization just yet. Especially in a laboratory setting, it can be difficult to implement green chemistry principles when designing all chemical reactions; the smaller scales are more forgiving, both in terms of cost and safety. However, as seen in the examples above, adopting these practices can have a huge positive impact in an industrial or manufacturing setting.
It is our responsibility to design reactions that are safe and sustainable, both with regard to the individual, the environment as well as the operating costs. With this in mind, applying the 12 principles of green chemistry should become second nature to us, from the initial planning of the reaction on the benchtop all the way to large-scale output.
Cover graphic: artist’s rendition of proline—an organic catalyst—forming a ‘recycle’ symbol by Melanie (@nanoclustering)
Reference
- Anastas, P., & Eghbali, N. (2010). Green chemistry: principles and practice. Chemical Society Reviews, 39(1), 301-312.
- Sawin, J. L., Sverrisson, F., Seyboth, K., Adib, R., Murdock, H. E., & Lins, C. (2014). Renewables 2014 global status report. Paris: REN21 Secretariat REN21.
- Hamed, I., Özogul, F., & Regenstein, J. M. (2016). Industrial applications of crustacean by-products (chitin, chitosan, and chitooligosaccharides): A review. Trends in Food Science & Technology, 48, 40-50.
- Hoffmann, R. W. (2006). Protecting-group-free synthesis. Synthesis, 2006(21), 3531-3541.
About the Author
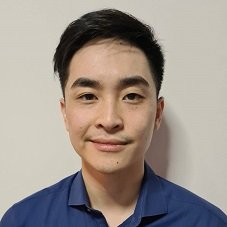
Sean is a consultant for clients in the pharmaceutical industry and is an associate lecturer at La Trobe University, where unfortunate undergrads are subject to his ramblings on chemistry and pharmacology.